Morphological Variation in the Seed of Gray Birch (Betula populifolia): The Effects of Soil-Metal Contamination
by Frank J. Gallagher1, Ildiko Pechmann2, Bernard Isaacson3, and Jason Grabosky1
1 Urban Forestry Program, Department of Ecology, Evolution, and Natural Resources, Rutgers University, 14 College Farm Road, New Brunswick, NJ 08901
2 Department of Biological Sciences, Rutgers University, 113 University Avenue, Newark, NJ 07102
3 Department of Forest and Wildlife Ecology, University of Wisconsin-Madison, 1630 Linden Drive, Madison, WI 53706.
Abstract
Few species of trees are known to colonize disturbed sites with the higher than normal concentrations of soil metals typical of urban brownfields. The patchy distribution and heterogeneous makeup of such soil contamination produces strong abiotic filters limiting recruitment from regional species pools. In the northeastern United States, one of the most successful species in these situations is gray birch (Betula populifolia). Our previous work indicates that gray birch dominates the study site within Liberty State Park, a known brownfield, and that its distribution could be positively correlated with increasing soil-metal loads. Our current study questions whether gray birch seeds collected from areas of various soil-metal loads have differing wing-loading rates, and thus varying dispersal effectiveness. In addition, we investigate whether seed viability is affected by soil-metal load. Such differences could rapidly alter gene flow within isolated urban assemblages. Our results indicate that gray birch seeds exhibit a broad range of wing-loading rates, caused by differences in the surface area and mass of the seeds. Wing-loading rates (seed weight/seed surface area) exhibited no significant relationship with soil-metal load, indicating that potential for seed distribution from areas of high soil-metal load has not been impaired. However, germination success exhibited a negative correlation with increasing soil-metal load—in fact, seeds from areas of high soil-metal loads generally failed to germinate. We conclude that metal tolerance in gray birch is probably the result of genetic variation maintained within the metapopulation.
Keywords: seed dispersal, rate of descent, metaliferous soil, assemblage structure, wing load
Introduction
Plant assemblage or redevelopment can be viewed as a process in which a series of filters allow for the selection of certain species from a regional pool (Weiher and Keddy 1995; Diaz et al. 1998). Typical filters include both abiotic and biotic limiting factors (Hobbs and Norton 2004). Of these, the effective seed dispersal and successful germination are critical to the distribution of plant assemblages. This is especially true in urban areas where open space is fragmented, making seed recruitment more difficult. Understanding the mechanics governing such filters helps to build a functional understanding of assemblage development and guild trajectories in the urban context.
Seed morphology generally correlates well with distribution strategies (Green 1980). Of the three vectors associated with seed dispersal (animal, water, and wind), wind-dispersed species tend to produce functionally similar seed structures that increase buoyancy in relationship to weight. While many species across a broad taxonomic range exhibit such structures, it has been suggested that diaspore architecture is the result of both functional and phyletic constraints (Matlack 1987).
Soil chemistry—and specifically, toxicity—are also known to have a significant impact on the development of woody species, especially those growing on mesic or xeric sites. In northern temperate deciduous forest, the pattern of stand development after disturbance is dependent on soil type variations at a very small scale (Liptzin and Ashton 1999). In urban landscapes, most soils have been compromised by human activity, especially those associated with industrial activities. Such soils typically contain trace metals, such as cadmium, copper, zinc, and lead, at higher than normal concentrations (Dudka et al. 1996). These elements are often adsorbed or occluded by carbonates, organic matter, Fe-Mn oxides, and primary or secondary minerals (Adriano 1986; Ross 1994). Since many metals in small concentrations are known to be essential for plant growth, the mechanisms for uptake are inherent, if somewhat species-specific: Most plants will tolerate or have a requirement for metals at concentrations within a particular range (known as the functional range). At concentrations above the functional range, plants can no longer maintain homeostasis, and metabolic functions are inhibited (Hilary and Wilkins 1987). Hence, soil-metal contamination can alter the structure and function of vegetative assemblages.
Gray birch (Betula populifolia) is a pioneer species that commonly invades old fields and burned-over or cleared land, and generally competes well in soils with low nutrient availability (Elias 1980). It has also been demonstrated that seed impingement (attachment to a substrate) and germination is greater in mineral soils with little leaf litter (Houle 1992). Gray birch often colonizes urban green space and, in our study area, is the dominant tree species (35% cover) (Gallagher et al. 2008). The tree produces small, winged seeds that are wind-dispersed.
In addition to variations in surface area and mass, which can alter seed distribution, there are several other mechanisms that help to explain the effectiveness of gray birch as an early colonizer of disturbed areas. In these fragmented landscapes, especially those isolated within urban settings, the distribution of seed at long distances maintains gene flow within the metapopulation (Cain et al. 2000). Wind-borne seed dispersal in plants tends to be biphasic: It behaves in accordance with the aerodynamic nature of seed when winds are under a specific velocity, but once that critical wind velocity is exceeded, the surface area and shape become less important, as the force of the wind is strong enough to carry the seed above the tree canopy. Extremely small and light seed, such as those of gray birch, do not require great wind velocity to be lifted above the canopy. Seeds pushed above the canopy tend to travel far greater distances than seeds that are released and remain within or below the canopy (Horn et al. 2001), and therefore show enhanced dispersal effectiveness.
Catkins of gray birch tend to be persistent, lasting well into the winter. The scales and seed tend to separate first at the tip of the catkin, proceeding gradually back to its base. An increase in a prevailing westerly wind correlates strongly with the staged release of seeds and also facilitates the increasing lift that results after the leaves are shed from the tree, as sparse canopies, such as those with little or no leaf mass, tend to have a more organized vertical eddy motion (Nathan and Katul 2005).
Our previous work demonstrated that soil-metal loads above a critical threshold concentration significantly affected primary productivity and yearly tree growth rates of gray birch (Gallagher et al. 2008). Yet, despite a decrease in production, gray birch dominated the areas we studied. The objectives of this study were to determine whether gray birch seeds collected from areas of high soil-metal load (above the defined TML threshold of 3.0) exhibited morphological differences that could influence wing loading and dispersal effectiveness. In addition, since gray birch preferentially colonized areas of high soil-metal load, we questioned whether seeds from these areas were equally viable. We hypothesized that these seeds would have similar if not improved dispersal ability and would exhibit comparable viability.
Materials and Methods
Study Area
Liberty State Park (LSP) is located (40° 42' 16 N, 74° 03' 06 W) on the west bank of Upper New York Bay in Jersey City, NJ, and is one of the most successful urban brownfield restoration initiatives in the state. The parkland was originally part of an intertidal mud flat and salt marsh that was filled for use as a rail yard. The fill materials consisted primarily of debris from construction projects and refuse from New York City, which were deposited between 1860 and 1919 to stabilize the surface. Between 1864 and 1967, the Central Railroad of New Jersey used the site as a rail yard for both freight and passenger service. Due to this industrial land use, higher than normal levels of soil metals are unevenly distributed throughout the site (Gallagher et al. In press). In the center of the park approximately 40 hectares remain undeveloped and, in spite of the contamination, various plant assemblages have colonized the area. These assemblages represent unique associations of both endemic and nonnative species that can be considered a by-product of the cultural events that have taken place during the past 150 years. The hardwood assemblage is dominated by gray birch (35% cover), and also contains significant numbers of eastern cottonwood (Populus deltoides) (16% cover) and trembling aspen (P. tremuloides) (14% cover).
Procedure
The total soil-metal load (TML) for the study area has been described elsewhere (Gallagher et al. In press). Soil samples were collected from 32 sites within the study area and examined for concentrations of arsenic (As), chromium (Cr), copper (Cu), mercury (Hg), lead (Pb), vanadium (V), and zinc (Zn) (Table 1). The soil samples were collected with a soil borer in triplicate at 1-meter intervals in a triangular pattern. The sampling depth corresponded with the depth of greatest root concentration determined with a hand spade. Soil samples were cleared of gravel and visible plant material (roots and leaves) and were then placed in clean, new polypropylene containers and stored at 4oC. In order to calculate the total soil-metal load (TML), the results were rank transformed according to Juang et al. (2001) and then summarized for each site (Gallagher et al. In press). The resulting index provided a way to evaluate the cumulative soil-metal load impact using a scale from 0 to 5, with 5 indicating the highest cumulative soil-metal concentrations (Figure 1). The threshold value of 3.0 was used as a benchmark for areas of high metal load, based on our previous work, which indicated that soil-metal loads above this level had a significant sublethal effect of metal-induced stress on both plant productivity and long-term growth.
Catkins were collected at six different sites within the study area on october 8, 2007. The six sites were chosen because they had a similar site index (tree size, soil grain size, and moisture content), were dominated by gray birch, and had been used in our previous studies to define TML. (Site identification numbers 14, 14-16, 18, 41, 43, and 48 were consistent with our previous studies.) We collected catkins from both the upper and middle sections of the tree canopy. Each tree's seeds were stripped from the catkins and thoroughly mixed, and approximately two hundred seeds (Table 2) from each sampled tree were randomly separated for examination. We measured the seeds at their broadest dimension to determine diameter, as both wing and germ surface area contributes to wing-loading rates (Figure 2). The seeds were segregated according to size at the 0.1-millimeter level. Since the rate of vertical descent and resulting lateral transport of wind-dispersed seed is a function of both size and weight, we then weighed the seeds, using a Sartorius F1800 balance. When seed weight was below 0.1 milligram, they were weighed in groups (with usually between 3 and 5 seeds per group). The results were then divided by the number of seeds weighed to determine individual seed weight.
Click image to enlarge
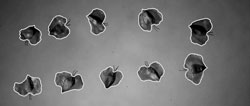
Figure 2: Approximately 200 seeds from each site were measured across their entire width, including both wing and germ, to determine radius. The surface area of each seed was calculated using the formula SA = πr2C. C is the mean ratio representing the difference between the surface area of 10 typical seeds, measured using ArcMap, and the surface area of a circle that encompassed those seeds.
We photographed ten randomly-chosen seeds from each site and calculated their surface areas using ArcMap (ArcGIS 2004) (Figure 2). We then calculated the surface area of each of the approximately 200 seeds from each site using the formula SA = πr2C, where C is a ratio representing the relationship between the mean surface area of ten photographed seeds from each site and the area of the circle resulting from their measured diameters. Wing-loading rates were then calculated by dividing seed mass by surface area.
All the seeds were cold-stratified in a refrigerator at 2°C for approximately 12 weeks. They were then sown in trays using a standard potting soil (1 part peat moss, 1 part coarse vermiculite, 2824 grams of pulverized lime, 807 grams Peters Uni-Mix Plus 10-5-10, 403 grams Rootshield G, and 33 grams Aquatrol) and placed on a bench in the Rutgers Floriculture Greenhouse on February 28, 2008. The greenhouse is heated, and the daily temperature ranged between 15 and 21°C. The seeds were watered by the greenhouse misting system and exposed to natural daylight. The seeds were sown in rows according to seed diameter. We made observations of the seeds once a day from March 3 to April 2, 2008 and considered the appearance of cotyledon leaves a benchmark for successful germination.
We used Tukey's HSD test for pair-wise comparisons to determine similarity between seed diameters and employed a two-tailed t-test to determine if the differences between seed diameter and mass above and below the TML threshold were significantly different. Descriptive statistical parameters for seed-diameter distribution, correlations between seed diameter and mass, and regressions between wing-loading rates and TML were calculated with Minitab (Minitab version, 2005) and SAS System (SAS 2003), with levels of significance set at a = 0.05. The Anderson-Darling normality test was used to assess seed diameter distribution within each sample.
Results
Seed diameter at each site varied by as much as 220% with far greater numbers in the midsize classes (Table 2). Seed diameter was normally distributed, as the Anderson-Darling Normality Test proved significant for all sites (p < .005 for 14,14-16, 18, 41; and p = .05 at site 48). Tukey analysis indicated that two sets of data (sites 41 and 18, 14 and 14-16) produced seeds of statistically similar size. Interestingly, the TML at sites 41 and 18 (0.85 and 2.85, respectively) were below the previously defined threshold for soil-metal tolerance of 3.0, whereas sites 14 and 14-16, which exhibited the smallest mean seed size, were above the threshold. Site 48 exhibited the largest mean seed size; however, the mean seed size at sites 41, 48, 43, and 18 varied by less than 17%. A two-tailed t-test between the sites above and below a TML of 3.0 was significant (t = 37.46, p < 0.01), indicating that seed size at sites 14 and 14-16 were significantly smaller than the rest of the samples.
In general it appears that seed diameter and mass do not scale evenly; Pearson correlations between seed diameter and mass within each site yielded inconsistent results (Table 3). Sites 41, 48, 14, and 14-16 yielded positive relationships, while 43 and 18 were negative. However, only two of the correlations were significant. At site 14-16, the site with the heaviest TML and smallest seed size, the correlation was strong (r = 0.82, p < .01). Site 48 also exhibited a weak but significant correlation (r = 0.46, p = .04).
Click images to enlarge
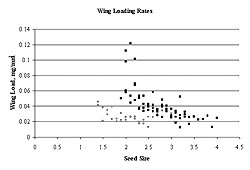
Figure 3: Wing-loading rates by seed size. Those sites above the TML threshold of 3.0 (sites 14 and 14-16, ) were slightly lower (F = 0.11, p < .01) than those below 3 (
). The major difference, however, is that sites above the TML threshold of 3.0 produce little seed with high loading rates.
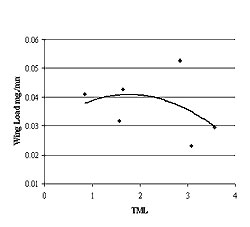
Figure 4: The relationship between the mean wing-loading rates and TML. The polynomial regression indicates that while a threshold model may be present, the regression was not statistically significant (r2 = 0.15, p = 0.55).
Wing-loading rates of seeds above the TML threshold of 3.0 were slightly lower than those below the threshold (F = 0.11, p < 0.01, Figure 3). While both of the sites above the TML threshold exhibited the lowest wing-loading rates, the trend of the quadratic polynomial regression (threshold model for metal tolerance) between mean wing-loading rate and TML was not statistically significant (r2 = 0.15, p = 0.55) (Figure 4).
The polynomial regression (Figure 5) between seed diameter and germination success was significant (r2 = 0.78, p < 0.01), which corroborates our work on productivity and tree growth (Gallagher et al. 2008). Germination rates ranged from a high of 19% at site 48 to a low of 1% at site 14-16. However, at site 41, the site that exhibited the lowest TML (TML = 0.85), only one of 195 seeds germinated, and these data were removed as outliers. Most significantly, germination rates and TML exhibit a strong negative correlation (r = -0.90, p = 0.04) (Figure 6).
Discussion
Seed diameter varied greatly at all sites. Site 14-16 exhibited the smallest range (1.4–3.1 centimeters), while site 18 exhibited the greatest range (1.9–3.2 centimeters). Tukey analysis and the two-tailed t-test clearly indicated that trees from sites where the TML was above the threshold of 3.0 produce seeds significantly smaller in diameter. This supports our previous observations of tree-growth trends (Gallagher et al. 2008). Interestingly, Tukey groupings also indicate differences in the remaining sites, and those where the TML was below the threshold also produced significant differences. However, since site 18 and 41 (also a Tukey grouping) had the broadest range in TML below the threshold of 3.0, and yet yielded almost identical seed diameters, it is unlikely that these differences are the result of either a stepwise or hormesis response to metal-induced stress.
Wing-loading rates within the sites ranged considerably; from 0.03 to 0.09 mg/mm2 at site 14-16 to between 0.02 and 0.12 at site 18, indicating that the potential for a considerable range in seed distribution exists at all sites. Wing-loading rates from sites with higher TML were slightly lower than at the other sites (F = 0.11, p < .01) (Figure 3). Mean wing-loading rates were more consistent, ranging from 0.03 at site 14-16, where TML was greatest, to 0.05 mg/mm2 at site 18, where our previous studies indicated greatest overall growth rates (Figure 4). We can conclude that trees from the area of high TML produce seeds with a range of loading rates, and such seeds could potentially respond to biphasic wind velocity principals in a similar manner to seeds from areas of lower TML.
Minor differences in seed structure have been correlated to considerable differences in dispersal performance (Dolan 1984; Matlack 1989; Peroni 1994). Higher wing loads produce faster rates of descent and thus decrease the potential for dispersion. On the other hand, lighter wing-loading rates, which can be produced either by decreased mass or increased wing size, can increase dispersal effectiveness. Our study indicates that, in general, seed diameter and mass in gray birch scale independently, producing a variety of wing-loading rates, which in turn increase the potential range of seed distribution. There were two exceptions: site 48, which yielded a marginally significant correlation (r = .48, p = .04); and site 14-16, which produced a strong positive correlation (r = .82, p < .01), however, the seeds from this site were found to be the lightest and the least viable in later testing. At site 14-16 an increase in wing diameter was more significant in determining wing load.
Seed surface area and mass are involved in distinct but related ecological functions (Matlack 1989). While seeds with lower wing-loading rates generally achieve greater dispersal distance, diaspores with higher wing-loading rates generally have heavier seeds, which produce more vigorous seedlings (Dolan 1984). These competing selective pressures have resulted in a broad spectrum of seed-dispersal strategies. Using paper birch (Betula papyrifera), Borkbum et al. (1965) suggested that seed mass and germination success were positively correlated. Similar results were found in this study, as the polynomial regression between germination success and seed diameter was strong (r2 = 0.78. p < 0.01) (Figure 5). In addition, the correlation between germination rate and TML was also strong (r = -0.90, p = 0.04), indicating that trees from areas of high TML—specifically sites 14 and 14-16—were unable to partition resources to seed production, and their seed was generally inviable. These results correlate well with metal-induced inhibition of seed germination found in several other species of plants (Munzuroglu and Geckil 2002), implying that areas of high TML are acting as a sink and not generally contributing to the spread of the species via sexual reproduction.
Kimmerer (1981) and Kalin and van Everdingen (1988) demonstrated that succession on metalliferous soil proceeds slowly, often remaining in the herbaceous stage for decades or centuries. In another study, the colonization and distribution of plant species on mine tailings from five Pb/Zn mines in China were still dominated (69.4% of total) by herbaceous species after twenty years of development (Li et al. 2007). These studies indicate that shifts in successional trajectories under soil conditions that produce metal-induced stress tend to favor herbaceous species. However, our previous investigation at this site and the work of Sanguer and Jetschke (2004), where birch was shown as an early colonizer of a former uranium-mining dump, indicate that the early arrival of tolerant woody species can alter the development. This current study furthers this finding, indicating that while seeds from trees within area of high TML can produce seed with a range of dispersal potential, they do not contribute to the migration of the species.
Understanding that high soil-metal load can affect the production of viable seed will help delineate where efforts to mitigate degraded soils will be required and where endemic assemblages can be maintained. Since such areas represent less than 20% of this particular site, mitigation efforts there can be significantly reduced.
Conclusion
This study confirms earlier findings that gray birch, like other birch species, produces small wind-borne seeds with considerable variation in surface area and mass. In addition, our data suggest that wing-loading rates are altered by changes in both size and weight of the seed, which show significant negative correlation with heavy soil-metal loads. Variability is further enhanced by the fact that seed surface area and mass do not scale evenly. This variability has the potential to produce a broad range of wing-loading rates well suited for colonization in the patchy habitat of urban areas.
Mean wing-loading rates were fairly consistent, indicating that seed from the area of high TML would respond to biphasic wind velocity principals similarly to the way in which seed from areas of lower TML do. However, since germination of the seed from areas of high TML proved to be far less effective, the success of gray birch trees in metalliferous soils must be due to a tolerance that is maintained in the metapopulation. Hence, the null hypothesis, that seed dispersal effectiveness and viability would not be significantly different above and below the critical TML of 3.0, was only partially correct. Finally, this study further justifies the implementation of a soil-mitigation plan to treat areas where the TML is above 3.0.
Acknowledgements
We wish to thank Peddrick Weis at the University of Medicine and Dentistry of New Jersey for analyzing soil samples and reviewing the paper. We also wish to thank Peter Smouse for his thorough edit of the manuscript. In addition, Gregory Dahle, Mike Gallagher, and Carolyn Haynes of the Urban Forestry Program at Rutgers University assisted with statistical analysis and seed measurements. The New Jersey Department of Environmental Protection and a McIntire-Stennis grant funded this study.